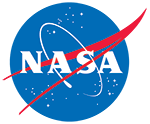
IceBridge PARIS L2 Ice Thickness, Version 1
Data set id:
IRPAR2
DOI: 10.5067/OMEAKG6GIJNB
This is the most recent version of these data.
Overview
This data set contains contains Greenland ice thickness measurements acquired using the Pathfinder Advanced Radar Ice Sounder (PARIS).The data were collected as part of Operation IceBridge funded campaigns.
Parameter(s):
ICE DEPTH/THICKNESS
Platform(s):
AIRCRAFT, P-3B
Sensor(s):
PARIS, RADAR ECHO SOUNDERS
Data Format(s):
ASCII
Temporal Coverage:
1 April 2009 to 2 May 2009
Temporal Resolution:
- Varies
Spatial Resolution:
- varies
- varies
Spatial Reference System(s):
WGS 84
EPSG:4326
Spatial Coverage:
N:
84
S:
60
E:
-11
W:
-73
Blue outlined yellow areas on the map below indicate the spatial coverage for this data set.
Data Access & Tools
A free NASA Earthdata Login account is required to access these data. Learn More
Documentation
Help Articles
How to Articles
Many NSIDC DAAC data sets can be accessed using the NSIDC DAAC's Data Access Tool. This tool provides the ability to search and filter data with spatial and temporal constraints using a map-based interface.Users have the option to
All data from the NASA National Snow and Ice Data Center Distributed Active Archive Center (NSIDC DAAC) is directly accessible through our HTTPS file system using Wget or curl. This article provides basic command line instructions for accessing data using this method.