A stormy May over the eastern Arctic helped to spread the sea ice pack out and keep temperatures relatively mild for this time of year. As a result, the decline in ice extent was slow. By the end of the month, several prominent polynyas formed, notably north of the New Siberian Islands and east of Severnaya Zemlya.
Overview of conditions
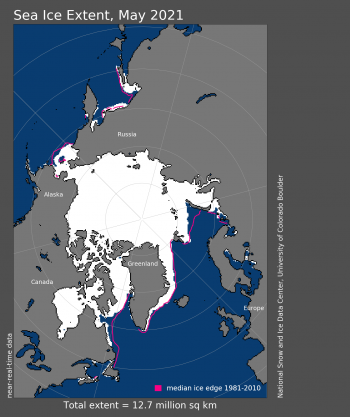
Figure 1. Arctic sea ice extent for May 2021 was 12.66 million square kilometers (4.89 million square miles). The magenta line shows the 1981 to 2010 average extent for that month. Sea Ice Index data. About the data
Credit: National Snow and Ice Data Center
High-resolution image
Arctic sea ice extent continued the slow pace of seasonal decline observed in April, leading to an average extent for May 2021 of 12.66 million square kilometers (4.89 million square miles). This was 740,000 square kilometers (286,000 square miles) above the record low for the month set in 2016 and 630,000 square kilometers (243,000 square miles) below the 1981 to 2010 average. The average extent for the month ranks ninth lowest in the passive microwave satellite record. The ice edge is near its average location most everywhere in the Arctic Ocean except in the Labrador Sea and east of Novaya Zemlya. Nevertheless, large polynyas have formed, notably north of the New Siberian Islands and east of Severnaya Zemlya. Open water areas have also developed near the coast in the southern Beaufort Sea and west of Utqiaġvik, Alaska (formerly Barrow). Overall, ice retreat during May occurred primarily in the Bering and Barents Seas, the Sea of Okhotsk and within the Laptev Sea.
Conditions in context
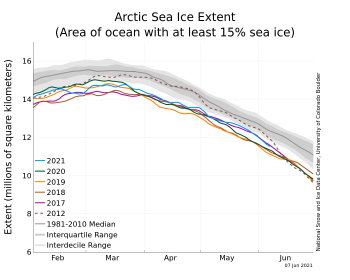
Figure 2a. The graph above shows Arctic sea ice extent as of June 7, 2021, along with daily ice extent data for four previous years and the record low year. 2021 is shown in blue, 2020 in green, 2019 in orange, 2018 in brown, 2017 in magenta, and 2012 in dashed brown. The 1981 to 2010 median is in dark gray. The gray areas around the median line show the interquartile and interdecile ranges of the data. Sea Ice Index data.
Credit: National Snow and Ice Data Center
High-resolution image
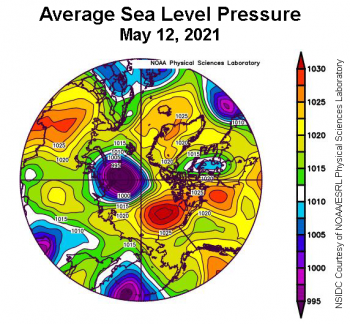
Figure 2b. This plot shows average sea level pressure in the Arctic in millibars on May 12, 2021. Yellows and reds indicate high air pressure; blues and purples indicate low pressure.
Credit: NSIDC courtesy NOAA Earth System Research Laboratory Physical Sciences Laboratory
High-resolution image
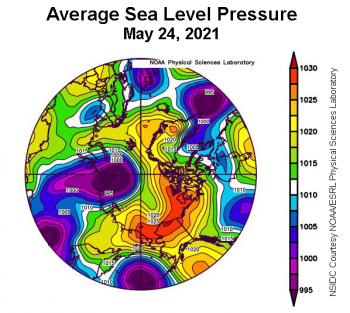
Figure 2c. This plot shows average sea level pressure in the Arctic in millibars on May 24, 2021. Yellows and reds indicate high air pressure; blues and purples indicate low pressure.
Credit: NSIDC courtesy NOAA Earth System Research Laboratory Physical Sciences Laboratory
High-resolution image
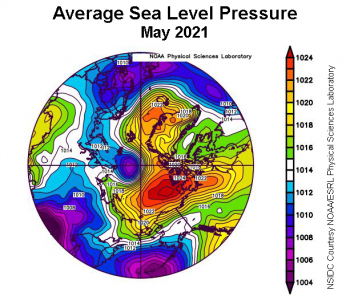
Figure 2d. This plot shows average sea level pressure in the Arctic in millibars for May 2021. Yellows and reds indicate high air pressure; blues and purples indicate low pressure.
Credit: NSIDC courtesy NOAA Earth System Research Laboratory Physical Sciences Laboratory
High-resolution image
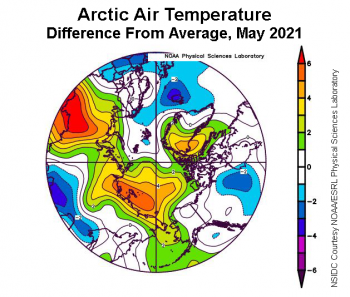
Figure 2e. This plot shows the departure from average air temperature in the Arctic at the 925 hPa level, in degrees Celsius, for May 2021. Yellows and reds indicate higher than average temperatures; blues and purples indicate lower than average temperatures.
Credit: NSIDC courtesy NOAA Earth System Research Laboratory Physical Sciences Laboratory
High-resolution image
The slow pace of sea ice loss this month (Figure 2a) can be explained in large part by a series of storms that migrated over the pole during May. The first storm split from a system over the Barents Sea and then slowly intensified over the central Arctic Ocean before reaching peak intensity (1007 hPa) north of Severnaya Zemlya on May 4. This was followed by another storm tracking northward from Europe, reaching peak intensity (sea level pressure of 987 hPa) over Severnaya Zemlya on May 12 and then joining with another storm that formed over Siberia on May 16 (Figure 2b). The strongest of the storms in terms of minimum central pressure (984 hPa) pressure, achieved on May 24, once again was located over Severnaya Zemlya and resulted from the merging of two systems moving in from the Barents Sea (Figure 2c).
As a result of the May storms, sea level pressure was lower than average by 6hPa centered just south of the pole at about 90 degrees E longitude. This was coupled with sea level pressure of 6 to 8 hPa above average over Greenland and the Canadian Arctic Archipelago extending into the northern Beaufort and Chukchi Seas (Figure 2d). Combined, this sea level pressure pattern fostered cold air spilling out of the Arctic Ocean into the North Atlantic and warm air flowing from the south over eastern Russia, leading to monthly averaged air temperatures at the 925 hPa level 1 to 4 degrees Celsius (2 to 7 degrees Fahrenheit) above average for this time of year over much of the Arctic Ocean, but up to 6 degrees Celsius (11 degrees Fahrenheit) above average along the coast of the Laptev and East Siberian Seas (Figure 2e). By contrast, temperatures were below average east of Greenland and around Svalbard. Wind patterns also explain the opening of the ice cover around Franz Joseph Land, the New Siberian Islands, and in the southern Beaufort Sea.
May 2021 compared to previous years
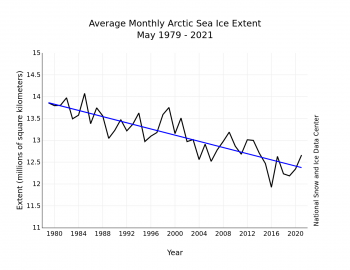
Figure 3. Monthly May ice extent for 1979 to 2021 shows a decline of 2.7 percent per decade.
Credit: National Snow and Ice Data Center
High-resolution image
Overall, the pace of ice loss was slower than average, leading to only the ninth lowest May extent during the satellite data record. Through 2021, the linear rate of decline for May sea ice extent, relative to the 1981 to 2020 average extent, is 2.7 percent per decade. This corresponds to 35,400 square kilometers (13,700 square miles) per year, about the size of the state of Maine. The cumulative May ice loss over the 43-year satellite record is 1.49 million square kilometers (575,000 square miles), based on the difference in linear trend values in 2021 and 1979. This is roughly twice the size of the state of Texas.
Capturing the break up in the Beaufort Sea
Visible wavelength imagery from the NASA Moderate Resolution Imaging Spectroradiometer (MODIS) provides the opportunity to track the ice breaking up in the southern Beaufort Sea this May; cloud cover was limited, offering decent views of the surface. Between April 25 and May 17, the pack ice started to move away from the landfast ice still attached to the coast, leading to open water and subsequent break up of the ice floes and partial break up of the landfast ice by mid-May. During the past winter, unusually high sea level pressure over the central Arctic Ocean resulted in unusually strong anticyclonic (clockwise) ice motion that drove a lot of fairly old ice from the central Arctic Ocean into the Beaufort Sea. Early break up of ice can enhance lateral and basal melt (at the underside of the ice) of the ice floes. This process can weaken the multiyear ice in the region and help to further deplete the Arctic of its multiyear ice. Large losses of multiyear ice in the region followed the unusually strong negative Arctic Oscillation winter of 2009 to 2010, which also featured a strong clockwise flow of the ice cover. More recently, analysis of Canadian ice charts by David Babb at the University of Manitoba suggests that between 2016 and 2020 on average 210,000 square kilometers (81,000 square miles) of multiyear ice now melts out each summer in the Beaufort Sea.
Are wavy jet stream winds wavier? Or not?
A new study takes a close look at an idea discussed several times in the Arctic Sea Ice News and Analysis (ASINA) reports—that Arctic Amplification, the observed strong warming in the Arctic region, driven in part by loss of Arctic sea ice, is affecting the shape and persistence of the jet stream. The polar front jet stream marks the boundary in the atmosphere between cold Arctic air and warmer mid-latitude air. Numerous studies have proposed that Arctic Amplification weakens the latitudinal temperature and atmospheric pressure gradient, manifested as a weaker and more sinuous jet stream. Since storms (low pressure systems) tend to form along the jet stream, weather in middle latitudes ought to become more variable, with large swings and more persistent patterns.
While the issue has long been controversial, the new study by James Screen, which was presented at the European Geophysical Union annual meeting in April, but has not yet been published, finds little evidence for this effect in climate model simulations and observations. In examining the past decade of observations, relationships that initially gave support to the idea have weakened. Even with far more open water conditions expected by 2050, the modeled effects of Arctic warming on the weather patterns at lower latitudes appear to be minor. The response is further obscured by the possibility of increased snowfall on Arctic land areas, creating cold regions that are not centered on the Pole. A separate new study by Jonathan Martin shows the polar jet has become slightly wavier and moved northward a bit, but maximum speeds in the jet are unchanged. The scientific debate on this issue is certain to continue.
Arctic sea ice thinning faster than expected
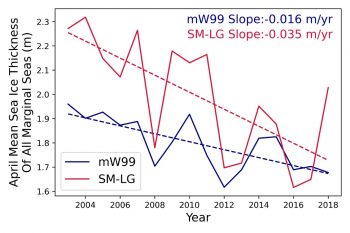
Figure 4. This plot shows average sea ice thickness in the Beaufort, Chukchi, East Siberian, Laptev, Kara, and Barents Seas in April 2004 to 2018 from the Environmental Satellite (Envisat) and CryoSat-2 radar altimeters, processed with the conventional snow product (modified Warren (1999) or mW99) and a new, dynamic snow product (from SnowModel-LG). The rate of decline is more than doubled when processed with SnowModel-LG, as the sea ice thickness inferred from snow cover diminishes.
Credit: R. Mallett, University College London.
High-resolution image
Satellites do not directly measure the thickness of sea ice. They measure the height of the ice surface above the ocean, termed the ice freeboard in the case of radar altimetry, or they measure the height of the ice plus the snow cover, in the case of laser altimetry. To convert these freeboards into total ice thickness requires knowledge of the depth and density of the snow cover atop the ice. Typically, a snow climatology based on snow depth observations collected several decades ago over multiyear ice is used. However, today’s Arctic mostly consists of smoother first-year ice, which tends to have a shallower snow pack than multiyear ice, allowing for deep snow accumulation around ridges. Further, delays in freeze-up and earlier melt onset in today’s warmer climate have reduced the time over which snow can accumulate on the ice. Both factors have resulted in a thinner snowpack than measured 20 years ago.
A new study published in The Cryosphere reveals that when using temporally varying snow depth and density estimates to convert ice freeboard to ice thickness, the ice is thinning at a faster rate in the Arctic marginal seas than previously believed (Figure 4). The time-varying snow depth is from a new data product, the SnowModel-LG, which is soon to be published at the NSIDC Distributed Active Archive Center (DAAC). It is based on coupling a sophisticated snow model with meteorological forcing data from atmospheric reanalysis systems and satellite-derived ice motion vectors. The study found that the rate of decline in ice thickness in the Laptev, Kara, and Chukchi seas was 70, 98 and, 110 percent faster, respectively, compared to previous estimates. As expected, the sea ice thickness variability also increased in response to interannually varying snow depth.
Antarctic notes
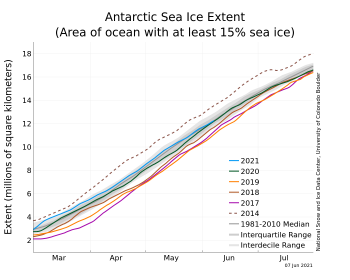
Figure 5a. The graph above shows Antarctic sea ice extent as of June 7, 2021, along with daily ice extent data for four previous years and the record low year. 2021 is shown in blue, 2020 in green, 2019 in orange, 2018 in brown, 2017 in magenta, and 2014 in dashed brown. The 1981 to 2010 median is in dark gray. The gray areas around the median line show the interquartile and interdecile ranges of the data. Sea Ice Index data.
Credit: National Snow and Ice Data Center
High-resolution image
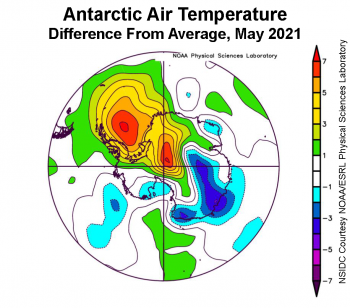
Figure 5b. This plot shows the departure from average air temperature in the Antarctic at the 925 hPa level, in degrees Celsius, for May 2021. Yellows and reds indicate higher than average temperatures; blues and purples indicate lower than average temperatures.
Credit: NSIDC courtesy NOAA Earth System Research Laboratory Physical Sciences Laboratory
High-resolution image
Antarctic sea ice extent grew at a slightly below-average pace in May, moving the overall extent from slightly above average to tracking the 43-year satellite record daily-extent average line (technically, the ‘median’ line) quite closely (Figure 5a). Sea ice extent was below average in the Weddell and Ross Seas, and slightly above average in the Bellingshausen and Amundsen Seas. In keeping with the sea ice trends, air temperatures for the month were well above average over the west-central Weddell Sea, about 7 degrees Celsius (13 degrees Fahrenheit) above average for the month (Figure 5b).
An embayment, or notch, in the ice edge in the eastern Weddell suggests that the processes that create the Maud Rise polynya were active, but at month’s end, the sea ice edge in that area (near 0 degree longitude and 68 degrees S latitude) had not enclosed the potential polynya region.
Further reading
Liston, G. E., P. Itkin, J. Stroeve, M. Tschudi and J. S. Stewart. 2020. A Lagrangian snow-evolution system for sea-ice applications (SnowModel-LG): part I–model description. Journal of Geophysical Research.-Oceans. doi:10.1029/2019JC015913.
Mallett, R. D. C., J. C. Stroeve, M. Tsamados, J. C. Landy, R. Willatt, V. Nandan and G. E. Liston. 2021. Faster decline and higher variability in the sea ice thickness of the marginal Arctic seas. The Cryosphere. doi:10.5194/tc-15-2429-2021.
Martin, J. E. 2021. Recent trends in the waviness of the Northern Hemisphere wintertime polar and subtropical jets. Journal of Geophysical Research-Atmospheres. doi:10.1029/2020JD033668.
Stroeve, J. C., J. Maslanik, M. C. Serreze, I. Rigor and W. Meier. 2011. Sea ice response to an extreme negative phase of the Arctic Oscillation during winter 2009/2010. Geophysical Research Letters. doi: 10.1029/2010GL045662.
Stroeve, J., G. Liston, S. Buzzard, L. Zhou, R. Mallett, A. Barrett, M. Tsamados, M. Tschudi, P. Itkin and J. S. Stewart. 2020. A Lagrangian snow-evolution system for sea ice applications (SnowModel-LG): part II–analyses. Journal of Geophysical Research-Oceans. doi:10.1029/2019JC015900.
Warren, S. G., I. Rigor, N. Untersteiner, V. Radionov, N. Bryazgin, Y. Aleksandrov and R. Colony. 1999. Snow depth on Arctic sea ice. AMS Journey of Climate. doi:10.1175/1520-0442.